Cell solutions: working at the small scale to tackle the global climate challenge
Climate change may be the biggest global challenge we currently face, but some of the most exciting solutions could come from working at the smallest scales of life. Caroline Wood takes a look at some of the new developments in cell science that could help us to slash our carbon emissions and achieve net zero.
ENERGY FROM ELECTROACTIVE MICROBES
A key challenge to decarbonisation is that our energy demands only keep growing, meaning we will need to develop new ‘fossil-free’ ways to power our societies. One potential solution could be provided by ‘electroactive microorganisms’: microbes that can exchange electrons with other microbial species, minerals, metals, or electrodes. These could both enhance already existing energy-generating processes that we rely on, or be applied in innovative new ways. It was recently discovered, for instance, that the microbes that perform anaerobic digestion of organic wastes forge electrical connections between each other, as Professor Derek Lovley (University of Massachusetts Amherst, USA) explains. “Converting wastes to methane requires a metabolic interaction between the bacteria that initially attack the waste, and archaea that produce the final methane product. We discovered that these two types of microbes cooperate by making electrical connections: the bacteria feed electrons to the archaea, which use the electrons to reduce CO2 to methane.” These connections are facilitated by protein monomers that are assembled into electronically conductive ‘protein nanowires’ that extend from the bacteria’s surface.1
Along with colleagues at Dalian University, China, Derek is researching whether this so-called direct interspecies electric transfer (DIET) can be accelerated and stabilised by adding conductive materials to the mix. Their approach was to use a mutant strain of bacteria in which genes for extracellular electron transfer had been deleted, meaning they were dependent on DIET from a wild-type strain to survive. Adding electrically conductive materials (such as carbon cloth and biochar) to the bacterial cultures accelerated the growth of the mutant strain, indicating greater DIET activity.2
“In particular, we found that conductive carbon materials with particle sizes larger than the microbe cells appeared to function similarly to an electrical grid by connecting the electron-donating partners with the electron-accepting partners. When the bacteria attach to the conductive material, they no longer have to make physical cell-to-cell connections,” says Derek. Preliminary studies suggest that these conductive materials significantly improve the rate of bacterial digestion for a broad range of organic matter, including dairy waste, kitchen waste, and dog food. “Ultimately, these results can be applied to increase the amount of waste materials we can treat using anaerobic digestion, greatly reducing our reliance on methane derived from fossil fuels ,” says Derek.
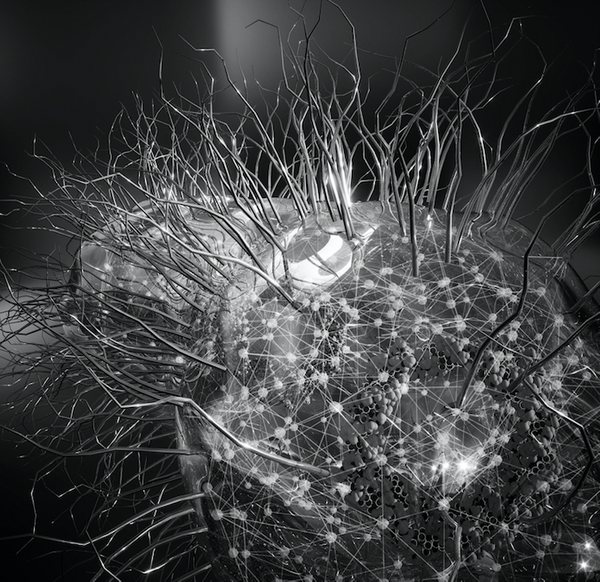
But Derek also intends to see electroactive microorganisms used in entirely novel applications. These include the Air-Gen (air-powered generator),3 a device developed by Derek and Massachusetts colleague Professor Jun Yao, which they claim can “literally make electricity out of thin air”. The simple device consists of two electrodes joined by a thin film of protein nanowires from the electroactive microbe Geobacter. “The concept came from an accidental discovery when we were experimenting with using protein nanowires in wearable electronic sensors. We found that without applying any electricity to the system, it began generating electricity itself,” Derek says.
The mechanism is currently unclear, but the group believe that when the protein wires absorb moisture from the air, a gradient of water molecules is established because only one end is exposed to the atmosphere. The charges associated with the nanowire monomers cause protons to be exchanged with the water molecules. This results in a proton gradient between the two electrodes, which ultimately introduces a voltage and electrical current.
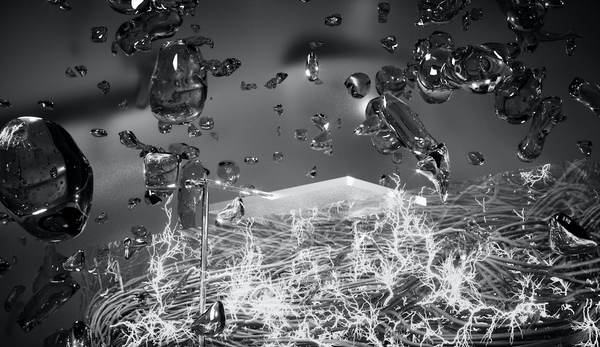
So far, the Air-Gen device has been demonstrated at laboratory scale with small devices with low energy requirements, including wearable biomedical sensors. Scaling it up into a device capable of producing substantial power will be challenging though, particularly because only a small volume of nanowires can currently be harvested from Geobacter. However, Derek and his colleagues have demonstrated proof of principle for producing commercial amounts of nanowires using an Escherichia coli strain with an introduced plasmid containing genes encoding nanowire monomers.4 “As an energy source, a large-scale Air-Gen device would have many advantages: it can produce electricity 24/7, it does not need sunlight or wind, it is low cost, and can even be used indoors,” says Derek.
REDESIGNING OUR DINNERS
Energy production isn’t the only thing that will need a drastic overhaul if we want to get on track to achieving net zero. It’s clear that population-level dietary change will also be necessary, particularly reducing meat consumption. Rearing livestock is a major source of greenhouse gas emissions, and one modelling analysis predicted that, under current trends, this sector alone would account for 49% of the emissions budget for limiting warming to 1.5°C by 2030.5 Cultured (or ‘lab-grown’) meat has been proposed as an alternative way to meet our growing protein demands, and the concept has already been demonstrated in various laboratories across the world. But can it really be scaled up to be a viable, economic, and ultimately environmentally sound option?
Marianne Ellis, a Professor of BioProcess & Tissue Engineering at the University of Bath, UK, certainly thinks so, and is currently researching how to make culturing meat as efficient as possible. “Once we have scaled the process up to commercial production, we can definitely aim at decarbonising the meat sector, ideally with a net zero carbon product,” she says. To create their lab-grown meat, Marianne’s team will use muscle stem cells taken from pigs or cows culled for food, although the team may move to harvesting cells from biopsies taken from living animals. These are cultured on a scaffold material, and supplied with glucose, amino acids, vitamins, and minerals. “We use various materials to construct scaffold, and these can be edible (for instance, decellularised grass), biodegradable, or reusable,” says Marianne.
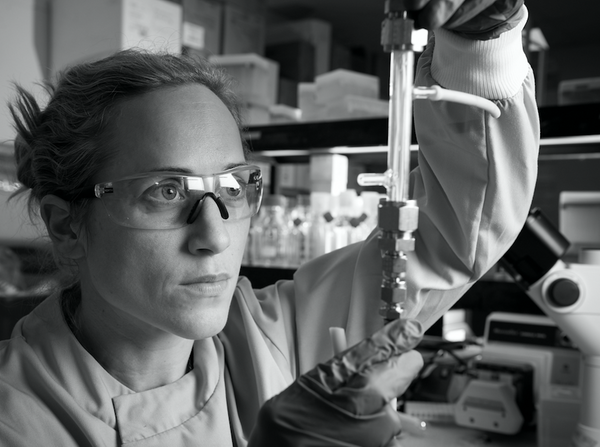
She cautions, however, that it will likely be at least 4–5 years before cultured meat arrives in UK supermarkets. A critical challenge is to bring down the currently prohibitive production costs. “We’re working to address the cost issue by looking at the various steps of the manufacturing process, in isolation and collectively, to find technical solutions to increase efficiency. For instance, using combined heat and power so that we utilise waste heat from one part of the process in another part,” she says. “But the main cost for lab-grown meat is the price of commercially available media which is priced for use in the biomedical industry; while this is not the core focus of our research, we are working with partners who are addressing this challenge.”
Some argue, however, that even when cultured meat production is optimised, it will never be as carbon-effective as plant-based protein sources. Indeed, with so many plant-based alternatives to meat now widely available, do we even need lab-grown meat? Some products seem indistinguishable from ‘the real thing’, such as the Impossible Burger, a soy-based burger that ‘bleeds’ like meat because of the addition of plant-based heme, and Loma Linda’s highly realistic vegan ‘Tuno & Mayo’.
But according to Marianne, lab-grown meat could still play an important role in offering choice and facilitating the transition to more plant-based diets. “Diversity of food supply is important for food security. A real advantage of cultured meat over rearing livestock is that it can be done anywhere in the world, including very hot and cold regions,” she says. “Also, those who have tried cultured meat —even a former meat producer—say that it smells and tastes like meat, so it could encourage meat eaters to substitute cultured meat into their diets when they may not substitute a plant alternative.”
PLUGGING THE GAPS IN CARBON STORAGE
In order to meet the Paris Agreement target to limit global warming to 1.5°C, it’s almost certain that reducing carbon emissions won’t be enough—we will also have to develop effective carbon capture and storage (CCS) technologies. One of the most promising of these is geologic carbon storage, where supercritical CO2 is injected into underground voids (such as former coal mines) for long-term storage. A critical problem, however, is that the upward pressure of CO2 gas can cause leaks, as Professor Andrew Mitchell (Aberystwyth University, UK) explains. “Although these wells are typically cemented after injection, the integrity of the seal often fails due to fracture formation and cracking. So we have been exploring whether we can instead seal these wells using microbial biofilms grown in situ.”
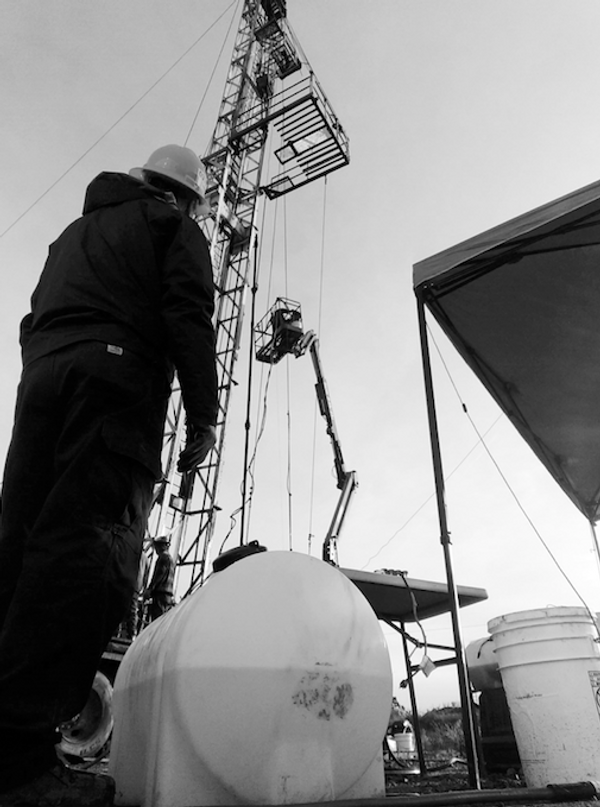
Working with colleagues from Montana State University, USA, Andrew realised that sealing these gaps with bacteria could potentially introduce another benefit: accelerated mineralisation of CO2 into stable solid rock . Carbonate ions from CO2 naturally react with calcium to form calcium carbonate, CaCO3. Certain microorganisms can enhance CaCO3 precipitation, for instance by bacterial ureolysis (the enzymatic hydrolysis of urea). This increases the surrounding pH, and hence the availability of CO32− and HCO3− ions.
The group’s initial laboratory studies used the ureolytic organism Sporosarcina pasteurii, suspended in synthetic brine containing urea and dissolved calcium ions.6 The vials had variable pressures of CO2 gas (produced using carbon-13 isotopes) applied to the headspace above the brine solution. As S. pasteurii hydrolysed urea, the dissolved calcium ions in the brine became completely precipitated as CaCO3. Moreover, the incorporation of carbon-13 carbonate ions into the precipitate rose as both the pressure of CO2 gas and the concentration of urea increased. “This shows that ureolysis effectively precipitated the CO2 that was initially in a gaseous phase over the brine,” says Andrew.
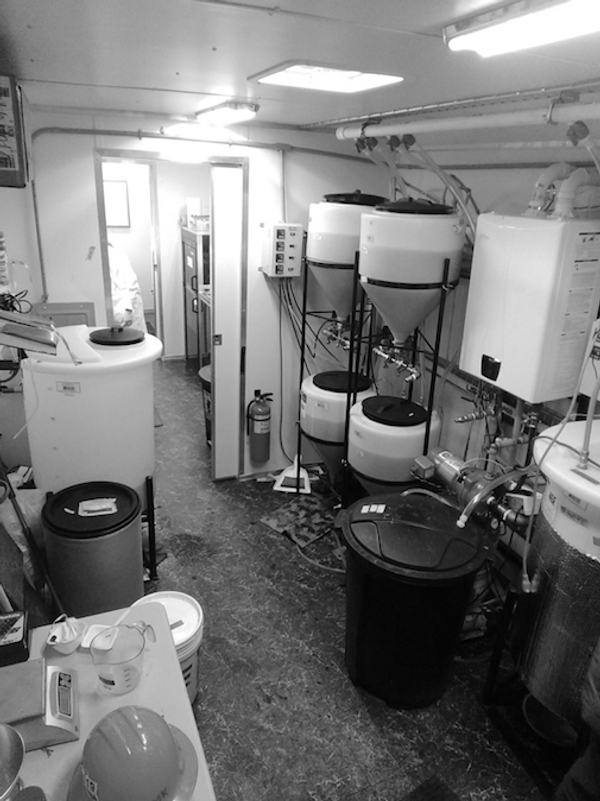
More recently, the team tested their system at an abandoned injection well in Alabama, where S. pasteurii demonstrated both rapid plugging and high integrity well sealing. Andrew admits, however, that targeted mineralisation and plugging may not be practical for large distances away from the injection well. Consequently, the team is collaborating with the Big Pit National Coal Museum in Wales to investigate whether native microorganisms could be stimulated to induce CO2 mineralisation alongside promoting groundwater heating and heat recovery .
“With wide-scale roll out of geologic carbon storage now under way in a number of countries, microbially induced CO2 mineralisation will likely have a significant impact on the long-term storage of CO2 underground and on reducing anthropogenic carbon emissions,” Andrew concludes.
TURNING CAST-OFF CLOTHES INTO CARBON CAPTURERS
An estimated 10% of greenhouse gas emissions are caused by the clothing and footwear industries, and under current trends these will use up around 26% of the world’s carbon budget by 2050.7 Besides being energy- and resource-intensive to produce, the vast majority of textiles are landfilled or incinerated at the end of their life, with only around 15% being recycled. But what if used textiles could be repurposed as carbon-capturing surfaces? Far from being ‘waste materials’, the fibres of used textiles could provide a perfect scaffold for photosynthetic microalgae that capture and store CO2 within their cells.
“Certain microalgae species are capable of very efficient photosynthetic carbon fixation, removing up to 1.83 kg of CO2 for every 1 kg of microalgae biomass produced,” says Dr Pichaya In-na (Chulalongkorn University, Thailand). “But conventional microalgae cultivation techniques based on suspending them in a solution limit CO2 transfer due to the relatively small gas/liquid interface.” In addition, these facilities require large areas of land and water, have high maintenance burdens, and are prone to contamination.
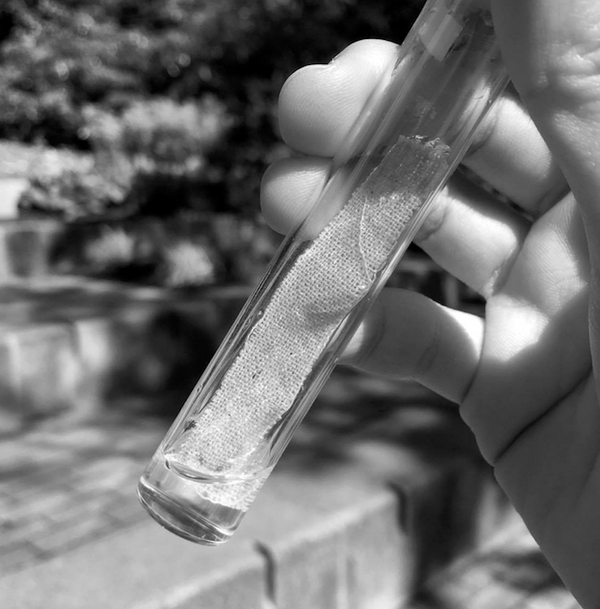
Working with colleagues Dr Jonathan Lee and Dr Gary Caldwell (Newcastle University, UK), Pichaya sought to address these problems using a ‘nature-inspired solution’. “We rationalised that by immobilising the algae on a scaffold, with minimal water present, we would increase contact with CO2 and therefore get substantial improvements in CO2 mass transfer,” she says. Concerned about the high volume of waste from the textile industries, they decided to investigate whether cotton and polyester materials could be suitable scaffolds.8 Strips of each material were submerged for 2 weeks in a suspension of the microalgae Chlorella vulgaris, chosen because of its resilience to a wide range of environmental conditions and the fact it is easy to grow and maintain.
After the cells had attached to the fibres, they were fixed in place with a hydrogel topcoat made of carrageenan, a polysaccharide extracted from seaweed. This protected the cells from drying out without affecting their photosynthetic performance. The samples were sealed within glass vials, into which a 5% CO2/air gas mixture was injected. Over the following month, the percentage of CO2 in each vial was quantified every 2 days by extracting 40 mL of gas and measuring its composition in a CO2 meter.
At the end of a month, the results showed that both biocomposites significantly outperformed the algae suspension, with cotton achieving the highest capture rates. “We found that the cotton-based living textiles had CO2 capture rates up to 11 times faster than algae grown in liquid cultures : 1.82 g of CO2 captured per gram of biomass each day, compared with 0.16 g,” says Pichaya. This difference was likely driven by two major factors. First, the mass transfer resistance for CO2 transfer would have been much smaller going from the gas phase to the cell cytoplasm (as in the biocomposites), compared with CO2 being transferred from a liquid suspension. Second, the CO2 concentration gradient would have been higher for the biocomposites, because most of the algal cells in the suspensions settled on the bottom, meaning they relied on CO2 diffusing through the bulk liquid water.
Remarkably, the biocomposite materials achieved these high carbon-capture rates despite the researchers not adding any additional water or nutrients from the start of the experiment. According to Pichaya, this indicates that biocomposite textiles could be a low-maintenance but resource-efficient strategy for carbon capture worldwide. “We were surprised at how long the biocomposites were able to function without further input of resources—almost a ‘hit the play button and forget’ type scenario,” she says.
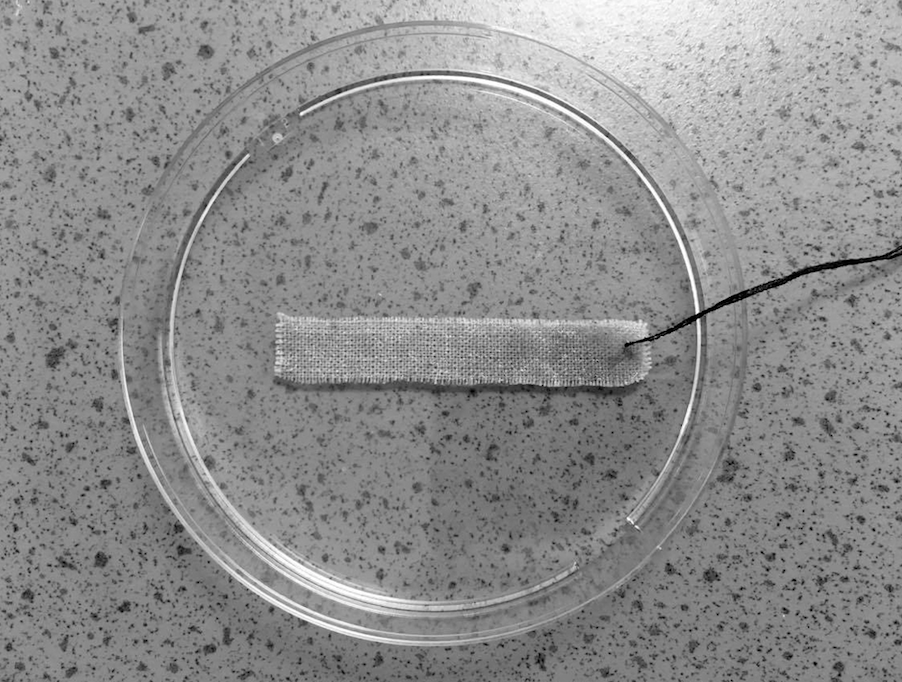
More development is needed, however, before these materials can be widely used. “Before we can go mainstream with carbon-capturing textile biocomposites, we need to make leaps forward to improve the robustness of both the cotton material and the hydrogel topcoat. Then we can aspire to have biocomposites that can function for years,” says Pichaya. Further work will look to optimise biocomposites for real-world applications, including capturing industrial sources of CO2. “It would be especially fitting to repurpose waste textiles and capture some of the textile industry’s own CO2 emissions ,” says Pichaya. “Nations such as India and Bangladesh that support substantial textiles industries are increasingly facing climate change-driven water crises. The low-water nature of our system therefore makes it ideal for such scenarios.”
References:
1. Liu X, Walker DJ, Nonnenmann SS, et al. Direct observation of electrically conductive pili emanating from Geobacter sulfurreducens. mBio 2021, 12: e02209-21.
2. Zhao Z, Li Y, Zhang Y, et al. Sparking anaerobic digestion: promoting direct interspecies electron transfer to enhance methane production. iScience 2020, 23: 101794.
3. Liu X, Gao H, Ward JE, et al. Power generation from ambient humidity using protein nanowires. Nature 2020, 578:.550–554.
4. Ueki T, Walker DJ, Woodard TL, et al. An Escherichia coli chassis for production of electrically conductive protein nanowires. ACS Synth Biol 2020, 9: 647–654.
5. Harwatt H. Including animal to plant protein shifts in climate change mitigation policy: a proposed three-step strategy. Climate Policy 2019, 19: 533–541.
6. Mitchell AC, Dideriksen K, Spangler LH, et al. Microbially enhanced carbon capture and storage by mineral-trapping and solubility-trapping. Environ Sci Technol 2010, 44: 5270–5276.
7. Ütebay B, Çelik P, Çay A. Textile wastes: status and perspectives. In: A. Körlü (ed.), Waste in Textile and Leather Sectors. London, IntechOpen, 2020.
8. In-Na P, Caldwell GS, Lee JG. Living textile biocomposites deliver enhanced carbon dioxide capture. Journal of Industrial Textiles 2021.